Understanding Germanium as a Semiconductor Material
Depicted as Ge on the periodic table, germanium presents an alluring enigma. Its role in shaping transistor technology is profound and mysterious. The intrigue of this semiconductor material stems primarily from its high charge carrier mobility traits that outshine those of silicon semiconductors. A distinctive attribute like no other, it grants swifter operation speeds and diminished energy consumption – qualities that render it a coveted choice for cutting-edge electronic contrivances.
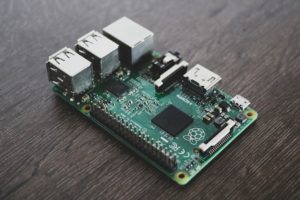
Moreover, germanium’s intrinsic attributes enable diffusion – a process where atoms navigate from areas teeming with concentration to zones sparsely populated within the semiconductor.
The mystery deepens when dopant materials are integrated into the matrix of germanium semiconductors. This step elevates their performance by altering their electrical conductive properties in peculiar ways. Introducing these dopants via controlled diffusion procedures can result in either n-type or p-type semiconductors; determined by whether electron-rich or electron-deficient atoms are incorporated respectively. Penetrating this complex interplay between germanium and dopant elements reveals precious insights into optimizing device performance.
However, venturing further into the labyrinth of germanium-based semiconductors uncovers challenges intertwined with opportunities. One significant hurdle refers to crafting reliable field-effect transistors using silicon-germanium alloys due to complications surrounding oxide stability and interface quality control parameters.
Yet hope glimmers through as ongoing experimental endeavors combined with theoretical research efforts illuminate possible solutions according to IEEE publications – carving pathways towards imminent advancements in this intriguing field.
It becomes evident that our journey exploring each atom’s potential power within Germanium has only just begun — an expedition promising enriched understanding should we continue delving deeper into its perplexing universe.
The Nature of Silicon-Germanium Semiconductors
The world of electronics has been gripped by the intrigue and promise of silicon-germanium (SiGe) semiconductors, revered for their distinctive electronic traits and high-mobility features. An alloy birthed from the union of silicon and germanium, SiGe is nurtured into existence via a method known as epitaxy. This process sees individual atomic layers meticulously deposited onto a crystalline substrate under tightly regulated conditions; it’s here that we see an interface between semiconductor material and its insulator emerge with defects kept to an absolute minimum.
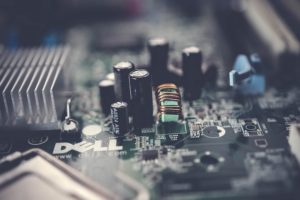
However, lurking within this intricate dance of scientific creation is the issue of dopant diffusion – a significant player in determining the performance adequacy of SiGe devices. These dopants are impurities willingly brought into play to tweak semiconductor properties yet they have a propensity for migration or diffusion through the lattice structure over time, leading to alterations in device behaviour. When it comes to SiGe alloys, various factors such as temperature fluctuations and ion concentration levels meddle in this diffusion process. It’s worth highlighting that these doping procedures can bestow certain coveted characteristics – like bandgap engineering for optimized field-effect transistor functionality – but they also bring with them challenges around passivation: neutralising those pesky dangling bonds with hydrogen atoms.
An array of low-temperature processes have been rigorously examined as potential solutions for curbing dopant diffusion within SiGe semiconductors. A host of IEEE published research indicates strong evidence that applying these cooler techniques during fabrication aids in maintaining superior control over distribution patterns within the materials – enhancing overall device efficiency reliability.
Yet science presses on relentlessly towards further advancements: integrating elements from group III-V into Silicon Germanium structures for cutting-edge CMOS applications demanding higher electron mobility than conventional silicon-based semiconductors can deliver.
Nonetheless,the task at hand remains challenging as there must be no compromise on other critical parameters such as thermal stability or compatibility with existing manufacturing workflows when introducing fresh elements into these elaborate systems.
Electron Mobility in Germanium and Its Impact on Device Performance
In the realm of semiconductors, Germanium’s (Ge) electron mobility assumes a pivotal role. Its growth process can sway its electronic structure and henceforth, the mobility of electrons within it. This becomes especially pertinent when strain induced during this process comes into play – affecting not just charge carrier distribution but also overall device performance. Furthermore, dangling bonds at surfaces or defects in germanium owing to impurity incorporation can scatter charge carriers, thereby negatively influencing electron mobility.
Yet these hurdles pave way for technological advancements in semiconductor technology. One such tactic is passivating Ge surfaces with an oxide layer to curb dangling bonds and reduce defect density. Additionally, alloying Ge with Silicon (Si) has shown promise; thus giving birth to Si-Ge semiconductors that amalgamate high electron mobility from Ge and superior thermal stability from Si – bolstering the functionality of field effect transistors used across various device applications.
Moreover, both experimental and theoretical studies have shed light on how incorporating III-V materials like gallium arsenide into germanium quantum well structures boosts ×10 electron mobilities versus pure germanium alone.
As we venture further into new territories for enhancing device performance through improved control over electron mobility methods like metal-oxide-semiconductor field-effect transistor (MOSFET) channel material engineering are making headway. For example, replacing native oxide layers on MOSFETs with germanium channels holds bright prospects for future semiconductor devices despite existing fabrication challenges tied up with achieving low-defect-density interfaces between III-V materials and silicon dioxide insulators.
Diffusion and Doping Procedures in Germanium Semiconductors
The semiconductor realm has long acknowledged the potentiality of germanium, a high-mobility element. Its intrinsic characteristics render it suitable for diverse applications. The diffusion process in Ge (germanium), involving the migration of germanium atoms within the crystal lattice structure, is shaped by elements such as temperature and concentration gradients. Concurrently, doping techniques often entail introducing impurities into superior quality germanium to alter its electrical attributes; aluminium oxide serves an excellent example due to its diffusivity.
Silicon technology has held sway in transistor manufacture; yet recent progressions have spurred a shift towards employing germanium and silicon-germanium blends owing to their superior electron mobility traits. Germanium-based semiconductors’ fabrication typically encompasses depositing thin layers onto substrates via procedures like molecular beam epitaxy (MBE). These deposited strata are subsequently subjected to thermal treatments fostering diffusion and facilitating more efficient integration of doped elements into the semiconductor’s matrix.
A critical challenge encountered during this procedure is reducing defects in Ge while securing optimal performance concurrently. In response, scholars have probed different tactics including using silicon dioxide or other high-k materials rather than conventional gate dielectrics like silicon cmos according to “Proceedings Of The IEEE”. Moreover, they’ve been analyzing how embedding a thin layer of crystalline silicon between the transistor channel — created from either unadulterated Germanium or Germanium-rich Silicon Germanium — and insulating layer could substantially mitigate interface trap density issues thereby boosting device performance considerably. Furthermore, researchers persistently explore inventive techniques for generating smoother interfaces between these layers – especially at atomic scales – thus enhancing overall device efficacy even further.
Theoretical and Experimental Approaches to Germanium Semiconductors
With a renewed zeal, the exploration of germanium as a semiconductor material has experienced an upswing. This revival is largely tied to its potential utilisation in advanced transistor designs within the semiconductor technology arena. A crucial factor that propels this interest is germanium’s high electron mobility, making it an ideal candidate for incorporation into devices such as metal oxide semiconductor field effect transistors (MOSFETs). Fascinatingly, this characteristic provides germanium with an edge over conventional silicon transistors and paves the way for improved device efficacy and performance.
From a theoretical perspective, much effort has been dedicated by researchers into deciphering Ge’s electronic structure. With an energy gap straddling around 0.2 eV between valence and conduction bands, it finds itself nestled among Group IV semiconductors – a category hosting elements like Silicon. There are ongoing attempts to devise models that can precisely predict these materials’ behaviour under assorted conditions. Furthermore, these substances are also being scrutinised in conjunction with silicon-germanium systems where they constitute intricate entities such as silicon on insulator (SOI) platforms.
On the empirical side of things, scientists utilise diverse diffusion and doping techniques to tailor-make properties of germanium semiconductors according to specific requirements. These procedures encompass introducing impurities into pure germanium aiming at altering aspects like charge carrier concentration and mobility etcetera which ultimately modifies its attributes significantly . It warrants mentioning that these explorations comprise both independent germanium components along with ones integrated within broader classes of materials in comprehensive semiconductor systems.
This manuscript falls under open access purview distributed conforming to Creative Commons Attribution license stipulations allowing unrestrained usage provided due credit is given for original work.
Comments are closed.